
Image credit: Plateresca/iStock/Getty Images Plus via Getty Images
The Art of Bacterial Warfare: Know Thy Enemy
A new European project investigates the antibiotic resistance microbiome in oyster culture regions and seeks to unravel how antibiotic resistance genes move in the surrounding habitats
About 2,500 years ago, Sun Tzu wrote in The Art of War, "If you know your enemy and know yourself, you need not fear the result of a hundred battles."1
These wise words are still relevant regarding the approach to treating escalating battles with bacterial infections. We are in an "arms race" with bacteria that continue to evolve mechanisms for evading the antibiotic compounds used against them. The arms race continues as we learn about our enemy. Antibiotics have served medical science for about 70 years, but bacteria have an edge over humans in this arms race. Time and evolution have enabled a vast repertoire of microbial artillery in the form of antibiotics—which are used to combat against competing bacterial and fungal species—as well as antibiotic resistance genes to fight back.
More recently (in evolutionary time scales), the fighting has been directed against humans who have coopted antibiotics for medicine. Microorganisms produce antibiotics for use against other microorganisms, with the exception of viruses and protozoa. The antibiotic resistance genes are a consequence of mutation and evolution of the genes that are the targets of those antibiotics (see below). Some antibiotics in use today are synthetic. Also, antibiotics should not be confused with antiseptics like iodine or bleach. Antiseptics can also be used for elimination of bacteria, but depending on the antiseptic (e.g., bleach), they cannot be taken internally since they create damage to the host cells, as well.
The war between bacteria and humans was a relatively one-sided affair, with bacteria usually winning the battles (e.g., the Black Plague), as discussed by B.B. Finlay.2 However, in the current context, we are focusing on antibiotic resistance genes, rather than the virulence genes and toxins that pathogenic bacteria may also produce. Until relatively recently, not enough was known about bacterial "enemies" to enable humans to mount a direct attack. The first natural antibiotic was discovered by Alexander Fleming in the 1930s while searching for the fabled "magic bullet"—a compound that would differentially stain microorganisms, but not animal cells, and also subsequently kill those microorganisms. (Note: Staining the cells was needed for identifying compounds that entered the bacterial cells. Once an effective killing compound was identified, this aspect of staining became subsidiary.)
After some bacterial cultures were accidentally contaminated by a mold from the genus Penicillium and impeded the growth of his studied bacterial culture, he found his "magic bullet," which he named penicillin. It is a member of the beta-lactamase class of antibiotics, which work by inhibiting cell wall synthesis. In 1939, scientists from Oxford University saw a need to develop penicillin for the soldiers who would inevitably fall ill during World War II. They demonstrated the effectiveness of penicillin for treating infectious disease in humans and, with collaboration from the U. S. Department of Agriculture (USDA), developed processes for large-scale production by 1945. The timing was a little late for penicillin use in World War II, but other wars were to follow—and so was the development of many other antibiotics, both natural and human-made. This was the beginning of the war between humans and bacteria.
Antibiotics can be classified by their chemical structure and also by the manner in which they attack and kill bacteria by interfering with the basic functions of cellular metabolism—i.e., cell wall synthesis, nucleic acid (DNA or RNA) synthesis, protein synthesis, and folic acid synthesis. Given that antibiotics are aimed at these functions, it can be anticipated that the ribosomes responsible for protein synthesis (and enzymes for the synthesis of cell wall components and nucleic acids) are the targets of antibiotic activities. In response to antibiotic attacks, the bacteria armed with a diverse gene pool have evolved appropriate adaptations, in the form of mutations in ribosomal constituents and biosynthetic enzymes, to prevent these assaults on cellular functions. The survivors of each attack pass on the successful genes to their future generations. Among these adaptations for avoiding antibiotic attack are specific efflux pumps that eject the antibiotic compounds from the cell (more on these below).
Treatment of infectious diseases was greatly improved after Fleming's discovery. Today, large quantities of antibiotics are used annually in medicine, agriculture, and aquaculture. Some of this use is for the enhancement of growth. Some use is merely preventive. The public perception of all antibiotic use being for therapeutic application is misleading. As an example, chicken is the most widely produced species of poultry globally, with over 90 billion metric tons of finished meat products produced annually.3 Various classes of antibiotics are implemented during the lifecycle of rearing from egg to "broiler-size" adult, which enhances the diversity of antibiotic residues associated with poultry production. The pharmaceutical industry-sponsored Animal Health Institute estimates that of the 17.8 million pounds of antimicrobials used for animals, 3.1 million pounds are used non-therapeutically; and in the case of some antibiotic compounds that resist metabolism, much of this can pass to the environment.4
In the case of antibiotics in feed for fish, it is estimated that 75 percent is released to the water.5 A further complication in the use of antibiotics in aquaculture is the difficulty in treating individual affected fish or shellfish, meaning that antimicrobials are used to treat entire populations with correspondingly high quantities of antibiotics.6 This can kill many bacteria, but the bacteria that survive can go on to fight the human–bacteria conflict another day. This is a driver of bacteria in environmental settings to become resistant to such compounds, creating a future where antibiotic use will be futile. It also facilitates the transfer of antibiotic resistances from agricultural pests to other organisms, including humans.7–14
The problem is widespread in agriculture generally. A study of "fire blight" in apple and pear trees considered the manner in which specific antibiotic resistance genes found their way to human nosocomial (hospital-acquired) infections in the northeastern U.S. Fire blight is a bacterial disease of apple and pear trees caused by Erwinia amylovora and is treated in open orchard settings by spraying significant quantities of streptomycin on affected orchards. As a spray, it can be expected to diffuse to outlying fields where other practices, such as livestock grazing, take place. Streptomycin-resistant strains of E. amylovora since 2002 have been identified in orchards of New York state, an area once thought to be a fire blight refuge for apple and pear trees.15 To understand how antibiotic resistance moves from one environment to another, nasal swabs and fecal smears of sheep that had been allowed to graze in fields before and after spraying with agriculturally relevant concentrations of streptomycin were analyzed for the presence of streptomycin-resistant E. coli.16 Swab and smear samples that were positive for streptomycin-resistant E. coli, collected from sheep after grazing on sprayed fields, had increased from 14.7 percent before spraying to 39.9 percent afterward. Similar studies have been approached in aquaculture settings,6,17,18,19 and the findings are clear that fish farm effluent is a significant point source for antibiotic resistant bacteria (ARB) (Figure 1).
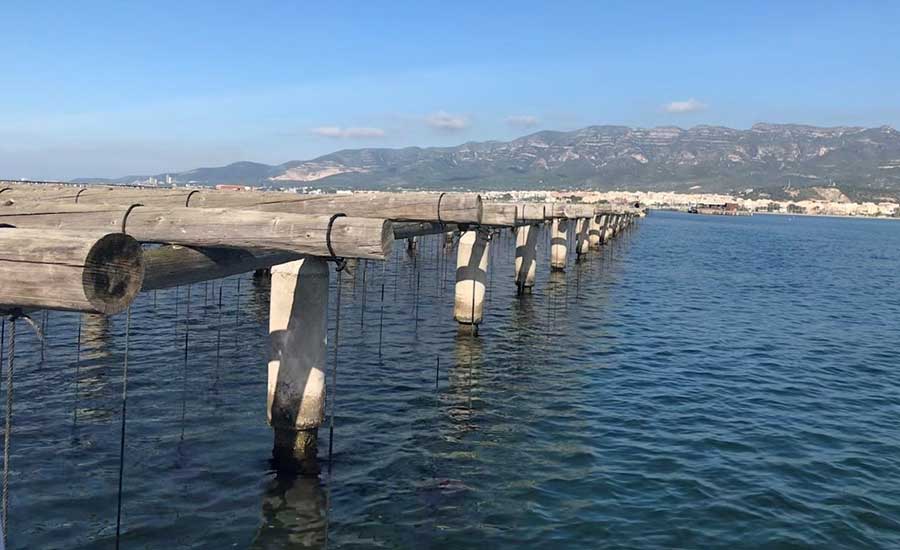
Scientists and legislators are taking notice. With the global threat of dissemination of antibiotic resistance and increasing medical treatment failures, the non-therapeutic use of antibiotics in animal production has been banned in some countries, mainly in Europe. Further interest in this topic was demonstrated in 2007 when the U.S. National Institute of Allergy and Infectious Diseases directed more than $800 million to antimicrobial resistance research alone.20 This is an example of financial resource allocation for human medicine; in areas related to animal health and production, expenditures are less.
However, advances are being made in aquaculture practices that will reduce the overuse of antibiotics, especially with the increased development of feed supplements for boosting growth and immune functionality.21 Various plant extracts or food processing byproducts, when incorporated into fish feed, can benefit the aquatic animals in ways that reduce the need for (or at least reduce the non-therapeutic uses of) antibiotics. Plants or their byproducts may contain phenolic, polyphenolic, alkaloid, quinone, terpenoid, lectine, and polypeptide compounds, many of which are bioactive alternatives to antibiotics.22 However, for filter-feeding organisms like shellfish (clams, mussels, and oysters raised in the open sea), these types of feed additives have less applicability. Furthermore, probiotics added to food will be another way to reduce the use of antibiotics. Probiotics are variously defined as living or nonliving, pure or mixed cultures of microorganisms provided in feed for the benefit of the host animal to promote growth, gut health, digestive capabilities, or immune status. Their use can have consequent problems (see below). In principle, however, the benefits to the health of the microbiome (the combined genetic material of all microorganisms in a particular environment), and therefore of the animal host, can be substantial.
Antibiotic Resistance Genes
Antibiotic resistance genes (ARGs) are DNA sequences that provide protection from the lethal effects of antibiotics on bacteria. They can be intrinsic to the bacterial genome or extrinsic genes acquired from other bacteria by mobile genetic elements. Bacteria that survive an antibiotic treatment will reproduce and move through the environment to spread these genes. The genetics of bacteria are very plastic with multiple mechanisms for creating genetic diversity including the transfer of DNA between cells vertically (across generations) and horizontally (among cells of the same generation), as well as between different species. These DNA sequences can move among bacterial cells as autonomous supplemental segments of the bacterial genome (plasmids, or mobile genetic elements), with varied mechanisms for generating new strains of ARB. Such supplemental genetic elements can be thought of as a suitcase. They are portable, transferable, and may carry different sets of genes—some for increased virulence, some for antibiotic resistance, and additional genes to provide protection from heavy metal or chemical intoxication.
In this way, bacteria are capable of rearranging ARGs as they associate with different environments or as conditions change in the environment. As new ARGs are acquired, they can accumulate in the genome plasmid or the host bacterial cell, thereby creating new strains of ARB and, ultimately, altering the overall microbiome. Among aquaculture-producing countries, frequently there are isolated ARB such as Vibrio spp., Aeromonas spp., Bacillus spp., Pseudomonas spp., Enterobacteriaceae, Streptococcus spp.—all groups that contain significant numbers of aquatic animal pathogens.23 Regarding the probiotic bacterial strains mentioned earlier, the extrachromosomal DNA fragments such as plasmids are one of the reasons that probiotics can be both a benefit and a risk. Some probiotic strains also harbor plasmids containing ARGs that can be passed to potentially pathogenic bacteria in the gut, converting a treatable bacterial infection into a more significant threat. This risk is more probable with live probiotic strains.24
The Comprehensive Antibiotic Resistance Database (CARD) lists approximately 5,000 different ARG sequences; the list continues to grow. These ARGs represent variants of normal genes with other functions that are targets of antibiotic activity. They have evolved within the bacterial kingdom for their own protection against other bacteria and fungi. This natural genetic variation is of benefit to bacteria in avoiding the actions of antibiotics.
For most antibiotics, there are many different antibiotic resistance genes that can have different mechanisms of action. However, we increase the abundance of ARGs through the misuse and overuse of antibiotics. The continued release to the environment of antibiotics as waste, combined with the chemical stability of specific antibiotics, creates persistent selective pressures for bacteria to retain ARGs, so there is an imperative to discontinue the release of antibiotic residue into the environment.
In countries with more developed economies and better government oversite of aquaculture regulations, some progress has been achieved. Less than six antibiotics are approved for aquaculture use in several EU countries and also in the U.S. By contrast, China and Vietnam allow the use of more than 30 different antibiotics for aquaculture and with less oversight on the doses or quantities used.23 Additionally, 90 percent of aquaculture global production is carried out in developing countries with similarly lax regulations.6 Global standards need to be established and enforced on all fronts (human medicine and agricultural uses) to reduce the environmental contamination by antibiotics in order to ameliorate the threat of antibiotic resistance. A positive step in this direction is the World Health Organization's AWaRe List for recommendations of antibiotic uses. It comprises a list of 87 antibiotics that are recommended for medical access; with additional lists of antibiotics to watch (147 in this group) as being more likely to elicit antibiotic resistance; and a reserve list (only 29) of antibiotics to be held for use only in such cases where multidrug resistance has been detected. Although more actions are needed, following such a guideline can be a deterrent to the proliferation of some ARGs.
The AWaRe list is merely one step in the right direction. The arms race with bacteria continues, and there are other fronts for attack in this battle. There are gaps in the knowledge of our enemy. It is clear that bacteria can transfer and acquire ARGs to improve their survival. It is also clear that bacteria can move among environmental compartments. Cutting off lines of transmission can be implemented only by knowing what those lines are, and where the vulnerabilities lie. In an aquatic setting, as compared to terrestrial habitats, the transfer among environmental compartments is difficult to control, especially at the microbial scale. More studies must be performed to identify routes of transfer and the sources and sinks of ARBs and ARGs to stop them at their source(s).
Although bacteria are simple, single-celled organisms, they actively protect themselves from environmental threats. The ARGs protect bacteria from antibiotics, whether administered by humans or excreted into the environment by other microorganisms. Bacteria also need protection from toxic substances in the environment like heavy metals (cadmium, mercury, lead, selenium, etc.), just as humans demand protection from exposure to such compounds in their environment. Among the different classes of ARGs are included efflux pumps to remove toxic compounds from the bacterial cell. Bacterial cell walls are perforated with protein gateways or channels, which can allow specific solutes to pass or be used to actively pump out toxic substances.
As an example, the genes for efflux pumps that remove heavy metal ions, rather than being an intrinsic part of the cell genome, are sometimes acquired in plasmids as a supplemental part of the genome acquired extrinsically for protection. When efflux pump genes are linked together in a mobile genetic element with other ARGs, the selection for one trait (such as antibiotic resistance) can lead to the acquisition of additional traits (such as resistance to toxic compounds), and vice versa. As an example, glyphosate inhibits the enzyme 5-enolpyruvylshikimate-3-phosphate synthase (EPSPS). This enzyme is commonly found in plants, but also in certain bacteria and fungi, although it is absent in mammals.25
The absence of EPSPS in mammals is the reason why glyphosate—a widely-used herbicide—is not considered toxigenic. However, the membranes of the gills of fish have been found to be damaged by glyphosate26 when exposed at sublethal doses, so it can have negative consequences for vertebrate organisms, as well. Glyphosate is used in the culture of genetically modified rice and corn that have been designed to resist the inhibition of EPSPS, and it is one of the most widely used herbicides globally. It is a form of phosphonic acid, with a chemical structure similar to fosfomycin, an antibiotic that is also derivative of phosphonic acid.
In E. coli, the presence of glyphosate increases the level of quinolone antibiotic resistance when bacteria are brought into contact with glyphosate at sublethal doses. Quinolones inhibit DNA repair enzymes, impeding DNA replication, while fosfomycin inhibits bacterial cell wall synthesis. The quinolone resistance in E. coli was found to be due to the overexpression of an efflux pump called AcrAB, which is a multidrug resistance marker.27 This finding indicates that the expression of quinolone resistance is genetically linked to the resistance to glyphosate. Although this is but one example, it shows how the presence of toxic/bactericidal chemicals or heavy metals can act as drivers of selection for ARGs and possibly also the generation of new ARB strains. For this reason, there is some urgency to understand the drivers behind genetic selection for ARGs. Only by acquiring this knowledge can better regulations be implemented to reduce the threat of antimicrobial resistance.
Additional studies from aquaculture show the prevalence of ARGs and the concomitant risks they may pose. Common infections in fish handlers working in aquaculture facilities include Aeromonas hydrophilia, Mycobacterium marinum, Streptococcus iniae, Vibrio vulnificus, and Photobacterium damselae, all of which are species of fish pathogens. Furthermore, fecal matter from Gilthead seabream (Sparus aurata) was found to contain extended-spectrum beta-lactamase (ESBL) resistance genes, including blaTEM-52 and blaSHV-12, as well as resistance genes against tetracycline, chloramphenicol, and sulfonamides.28,29,30 This is not limited to fish; in a study from Southeast Asia in shellfish, it was found that Vibrio parahemolyticus strains from oysters, although lacking TDH and TRH toxins typical of virulent strains, had multiple drug resistances.
Moreover, when resistance to heavy metals was examined, the majority of the Vibrio isolates from oysters displayed resistance to Ba²⁺ (98.3 percent), Co³⁺ (28.8 percent), Cd²⁺ (16.9 percent), and Cu²⁺ (13.6 percent). Additional analysis revealed that tolerance to heavy metals was most prevalent in the V. parahaemolyticus strains with more than two antibiotic resistance phenotypes.31 More studies like these are needed to understand the role that oysters, as shellfish that are often eaten raw, might play in spreading ARBs from the sea to humans. This is the premise and context of One Health: giving the health of the environment and all of its inhabitants, including humans, the same level of importance.
Some toxic metals, like arsenic, are found naturally in the minerals of the soil and can contaminate, at significantly high levels, water sources used by humans. This scenario is prevalent in some regions of the Indian subcontinent, for example. The natural abundance of toxic elements in the local geology cannot be easily controlled. Moreover, identifying the human origins of potential bactericidal compounds can facilitate identifying the sources and sinks that create the drivers for the selection of ARGs. When the toxic compounds or metals originate from human activities and drive the spread of antibiotic resistance, considerations must be made to limit the quantities and dosages sold and limit the conditions for use and disposal to avoid the co-selection of antibiotic resistance. Such compounds, and the ARBs and antibiotic residues in treated and untreated wastewater, eventually enter the seas, where they can influence the selection and genetic modification of bacteria inhabiting the ocean. Once incorporated into the collective metagenome of the littoral region, the ARGs can also move over longer distances by potential transportation of ARBs (and antibiotic residue) along the coastline in ship ballast water, as biofilms on boats, attached to cells in microalgae blooms, and in the microbiota of fish and marine bird feces, among other pathways (Figure 2).

Know Thy Enemy
A large commercial market for a particular food product creates a corresponding interest in problem-solving to benefit the efficiency and profit margin of that sector. Chicken, pig, and cattle production have significant commercial lobbies in national governments to support the financial investment in the development of those sectors. In the review by Watts et al.,6 aquaculture systems are described as "…containing high numbers of diverse bacteria, which exist in combination with the current and past use of antibiotics, probiotics, prebiotics, and other treatment regimens," thus designating aquaculture regions as "…genetic hotspots for gene transfer."
Routes of ARG transfer have been studied in finfish aquaculture; although, shellfish aquaculture is somewhat distinct in practice and comprises only a fraction of the commercial consumer market, and is less intensively studied. Shellfish culture falls behind finfish culture in terms of financial investment, and there is little known about the sources, sinks, and routes of transfer of ARGs in the unique setting of shellfish culture. This industry is always located nearshore, where the exposure to effluent varies by location and season. All anthropogenic effluents are potential drivers, but which ones can be controlled and how they are to be regulated cannot be known until they are identified. While significant effort is expended for the interests of finfish aquaculture, financial resources for shellfish culture are much more limited.
Interest in the growing proliferation of antibiotic resistance is gaining traction on multiple fronts, as evinced by new regulations and allotments in research funding. New research projects, as well as proposals to be funded, will help fill knowledge gaps and facilitate curbing the proliferation of ARGs. The risks to health were discussed in a recent article.32 As one example of efforts to better understand the role of shellfish in the spread of ARGs, project SPARE-SEA (environmental Spread and Persistence of Antibiotic REsistances in Systems Exposed to oyster Aquaculture), with €1.38 million in financing from the European Commission's Joint Program Initiative for Aquatic Pollutants, is studying the biodiversity of ARGs associated with oyster culture systems in Western Europe. Outcome from project SPARE-SEA will provide a better picture of what ARGs exist in the aquatic environments where oysters are cultured along the Western European coast, how they may have arrived in this habitat, what "drivers" increase their abundance, and what risks they may pose to the environment and to human and animal life.
Oysters are the focus of the project, in part because these animals are most likely to support the transmission of live bacteria to human hosts since they are frequently eaten uncooked; also, the watery medium of growth assists the transfer of bacteria among various aquatic hosts associated with oysters. The SPARE-SEA project, launched in 2021, is a collaboration of institutes from Germany (Alfred Wegner Institute), France (CNRS, L'Institut Français de Recherche pour l'Exploitation de la Mer), Spain (Institute for Research and Technology of Agro-alimentary Products), and Italy (University of Genoa and National Research Council of Italy) that is combining biochemical, microbiological, and genetic analyses of samples from each oyster-growing country.
In addition to oysters, the samples studied from different environmental sites in each country will include water, sediment, other shellfish species besides oysters, as well as additional invertebrates found in proximity to oyster culture facilities/sites. The genomic approaches used will identify potentially emerging ARGs that may pose distinct risks, and culture-dependent analysis of samples will help in identifying which bacteria are most likely to represent emerging pathogens originating from oyster aquaculture. The totality of the work should assist in identifying the drivers of selection for antibiotic resistance and some routes of transfer of ARGs among environmental segments of oyster-growing regions of Western Europe. With this knowledge, proper legislation can be formulated for securing a more healthy aquatic environment for all aquatic resource uses. The data that is acquired will improve our knowledge of our enemy as the arms race between bacteria and humans continues.
Acknowledgments
The author would like to thank the European Commission and additional national funding agencies for providing funds in the frame of the collaborative international consortium SPARE-SEA, financed under the 2020 AquaticPollutants Joint call of the AquaticPollutants ERA-NET Cofund (GA No. 869178). This ERA-NET is an integral part of the activities developed by the Water, Oceans, and AMR JPIs.
References
- Tzu, Sun. "On the Art of War." Translated by Lionel Giles. London: Luzac and Company, 1910.
- Finlay, B.B. "Stopping Infections: The Art of Bacterial Warfare." Scientific American. February 1, 2010. https://www.scientificamerican.com/article/the-art-of-bacterial-warfare/.
- Agyare, C., V. Boamah, and C. Osei. "Antibiotic Use in Poultry Production and Its Effects on Bacterial Resistance." Antimicrobial Resistance: A Global Threat. Yashwant Kumar, Ed. London: IntechOpen, 2018. https://www.intechopen.com/chapters/62553.
- Mellon M., C. Benbrook, and K.L. Benbrook. "Hogging It!: Estimates of Antimicrobial Abuse in Livestock." Union of Concerned Scientists. January 2001. https://www.ucsusa.org/resources/hogging-it-estimates-antimicrobial-abuse-livestock.
- Burridge, L., J.S. Weis, F. Cabello, J. Pizarro, and K. Bostick. "Chemical use in salmon aquaculture: A review of current practices and possible environmental effects." Aquaculture 306, no. 1 (2010): 7–23.
- Watts, J.E.M., H.J. Schreier, L. Lanska, and M.S. Hale. "The Rising Tide of Antimicrobial Resistance in Aquaculture: Sources, Sinks and Solutions." Marine Drugs 15 (June 2017): 158. https://pubmed.ncbi.nlm.nih.gov/28587172/.
- Carattoli, A. "Animal reservoirs for extended spectrum beta-lactamase producers." Clinical Microbiology and Infection 14 (2008): 117–123. https://pubmed.ncbi.nlm.nih.gov/18154535/.
- Depoorter, P., D. Persoons, M. Uyttendaele, P. Butaye, L. De Zutter, K. Dierick, L. Herman, H. Imberechts, X. Van Huffel, and J. Dewulf. "Assessment of human exposure to 3rd generation cephalosporin resistant E. coli (CREC) through consumption of broiler meat in Belgium." International Journal of Food Microbiology 159 (2012): 30–38. https://pubmed.ncbi.nlm.nih.gov/22938836/.
- Mayrhofer, S., P. Paulsen, F.J.M. Smulders, and F. Hilbert. "Antimicrobial resistance in commensal Escherichia coli isolated from muscle foods as related to the veterinary use of antimicrobial agents in food-producing animals in Austria." Microbial Drug Resistance 12 (2006): 278–283. https://www.liebertpub.com/doi/10.1089/mdr.2006.12.278.
- Silbergeld, E.K., J. Graham, and L.B. Price. "Industrial food animal production, antimicrobial resistance and human health." Annual Review of Public Health 29 (2008): 151–169. https://pubmed.ncbi.nlm.nih.gov/18348709/.
- Srinivasan, V., H.- M. Nam, A.A. Sawant, S.I. Headrick, L.T. Nguyen, and S.P. Oliver. "Distribution of tetracycline and streptomycin resistance genes and class 1 integrons in Enterobacteriaceae isolated from dairy and nondairy farm soils." Microbial Ecology 55 (2008): 184–193. https://pubmed.ncbi.nlm.nih.gov/17701242/.
- Stine, O.C., J.A. Johnson, A.K. Keefer-Norris, K.L. Perry, J. Tigno, S. Qaiyumi, M.S. Stine, and J.G. Morris, Jr. "Widespread distribution of tetracycline resistance genes in a confined animal feeding facility." International Journal of Antimicrobial Agents 29 (2007): 348–352. https://pubmed.ncbi.nlm.nih.gov/17287111/.
- Van Boxstael, S., K. Dierick, X. Van Huffel, M. Uyttendaele, D. Berkvens, L. Herman, S. Bertrand, C. Wildemauwe, B. Catry, P. Butaye, and H. Imberechts. "Comparison of antimicrobial resistance patterns and phage types of Salmonella Typhimurium isolated from pigs, pork and humans in Belgium between 2001 and 2006." Food Research International 45 (2012): 913–918. https://www.sciencedirect.com/science/article/abs/pii/S0963996911003413.
- Zirakzadeh, A. and R. Patel. "Epidemiology and mechanisms of glycopeptide resistance in enterococci." Current Opinion in Infectious Diseases 18 (2005): 507–512. https://pubmed.ncbi.nlm.nih.gov/16258324/.
- Russo, N.L., T.J. Burr, D.I. Breth, and H.S. Aldwinckle. "Isolation of Streptomycin-Resistant Isolates of Erwinia amylovora in New York." Plant Disease 92, no. 5 (2008): 714–718. https://pubmed.ncbi.nlm.nih.gov/30769587/.
- Scherer, A., H.-R. Vogt, E.M. Vilei, J. Frey, and V. Perreten. "Enhanced antibiotic multi-resistance in nasal and faecal bacteria after agricultural use of streptomycin." Environmental Microbiology 15. no. 1 (2013): 297–304. https://pubmed.ncbi.nlm.nih.gov/23157680/.
- Jo, H., S. Raza, A. Farooq, J. Kim, and T. Unno. "Fish farm effluents as a source of antibiotic resistance gene dissemination on Jeju Island, South Korea." Environmental Pollution 276 (2021): 116764. https://doi.org/10.1016/j.envpol.2021.116764.
- Penders, J. and E.E. Stobberingh. "Antibiotic resistance of motile aeromonads in indoor catfish and eel farms in the southern part of The Netherlands." International Journal of Antimicrobial Agents 31 (2008): 261–265.
- Jacobs, L. and H.Y. Chenia. "Characterization of integrons and tetracycline resistance determinants in Aeromonas spp. isolated from South African aquaculture systems." International Journal of Food Microbiology 114 (2007): 295–306.
- Landers, T.F., B. Cohen, T.E. Wittum, and E.L. Larson. "A review of antibiotic use in food animals: Perspective, policy, and potential." Public Health Reports 127, no. 1 (2012): 4–22. https://pubmed.ncbi.nlm.nih.gov/22298919/.
- Dawood, M.A.O., S. Koshio, and M.A. Esteban. "Beneficial roles of feed additives as immunostimulants in aquaculture: A review." Reviews in Aquaculture 10 (2018): 950–974. https://onlinelibrary.wiley.com/doi/abs/10.1111/raq.12209.
- Harikrishnan, R., C. Balasundaram, and M.S. Heo. "Impact of plant products on innate and adaptive immune system of cultured finfish and shellfish." Aquaculture 317, no. 1 (2011): 1–15.
- Hossain, A., Md. Habibullah-Al-Mamun, I. Nagano, S. Masunaga, D. Kitazawa, and H. Matsuda. "Antibiotics, antibiotic-resistant bacteria, and resistance genes in aquaculture: Risks, current concern, and future thinking." Environmental Science and Pollution Research 29 (2022): 11054–11075. https://doi.org/10.1007/s11356-021-17825-4.
- Gueimonde, M., B. Sánchez, C.G. de los Reyes-Gavilán, and A. Margolles. "Antibiotic resistance in probiotic bacteria." Frontiers in Microbiology 4 (July 2013). https://doi.org/10.3389/fmicb.2013.00202.
- Raoult, D., L. Hadjadj, S.A. Baron, and J.-M. Rolain. "Role of glyphosate in the emergence of antimicrobial resistance in bacteria?" Journal of Antimicrobial Chemotherapy 76, no. 7 (2021): 1655–1657, https://doi.org/10.1093/jac/dkab102.
- Braz-Mota, S., H. Sadauskas-Henrique, R.M. Duarte, A.L. Val, and V.M.F. Almeida-Val. "Roundup® exposure promotes gills and liver impairments, DNA damage and inhibition of brain cholinergic activity in the Amazon teleost fish Colossoma macropomum." Chemosphere 135 (September 2015): 53–60. https://doi.org/10.1016/j.chemosphere.2015.03.042.
- Aghamali, M., M. Sedighi, A. Zahedi, A. Bialvaei, N. Mohammadzadeh, S. Abbasian, Z. Ghafouri, E. Kouhsari. "Fosfomycin: Mechanisms and the increasing prevalence of resistance." Journal of Medical Microbiology 68, no. 1 (2019): 11–25. https://pubmed.ncbi.nlm.nih.gov/30431421/.
- Sousa, M., C. Torres, J. Barros, S. Somalo, G. Igrejas, and P. Poeta. "Gilthead seabream (Sparus aurata) as carriers of SHV-12 and TEM-52 extended-spectrum beta-lactamases-containing Escherichia coli isolates." Foodborne Pathogens and Disease 8 (2011): 1139–1141.
- Haenen, O.L.M. "Bacterial infections from aquatic species: Potential for and prevention of contact zoonoses." Revue Scientifique et Technique 32 (2013): 497–507. https://pubmed.ncbi.nlm.nih.gov/24547653/.
- Rivas, A.J., M.L. Lemos, and C.R. Osorio. "Photobacterium damselae subsp. damselae, a bacterium pathogenic for marine animals and humans." Frontiers in Microbiology 4 (2013): 283.
- Kang, C.H., Y. Shin, H. Yu, S. Kim, and J.S. So. "Antibiotic and heavy-metal resistance of Vibrio parahaemolyticus isolated from oysters in Korea." Marine Pollution Bulletin 135 (2018): 69–74. https://pubmed.ncbi.nlm.nih.gov/30301087/.
- Kijewska, A., A. Koroza, K. Grudlewska-Buda, T. Kijewski, N. Wiktorczyk-Kapischke, K. Zorena, K. and Skowron. "Molluscs—A ticking microbial bomb." Frontiers in Microbiology 13 (2023): 1061223. https://pubmed.ncbi.nlm.nih.gov/36699600/.
Karl B. Andree, Ph.D., obtained his doctorate in microbiology from the University of California–Davis. He works as an investigative researcher for the Institute for Research and Technology of Agro-Food Products (IRTA) in Spain and is a participating member of the SPARE-SEA consortium.